Stefania Gori, peering beyond the Standard Model
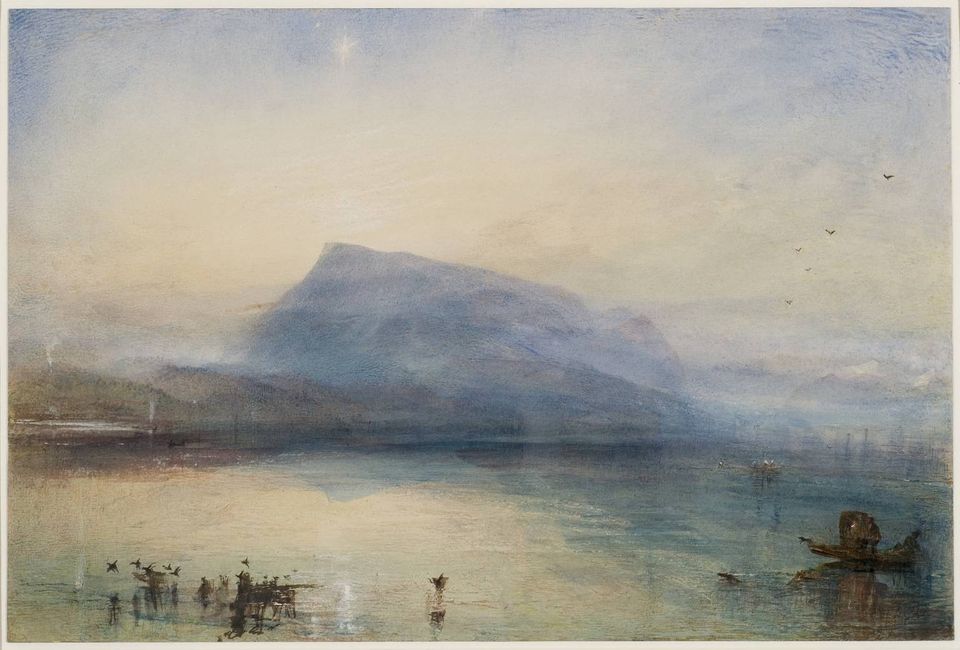
"If you just think about the Standard Model, the Higgs is the only particle that is distinguishing between the several fermion generations."
Right after the Muon g−2 results came out, I wanted to get a sense of how theorists were taking in the news that the anomaly was alive and well. I spoke with Stefania Gori, a theorist at the University of California, Santa Cruz. Stefania works on an impressively wide variety of BSM phenomenology, from exotic Higgs models to axions to rare pion decays. Beyond her own research, Stefania does outreach for women in physics and enjoys traveling.
(Note: This interview was conducted 4/9/21. It has been edited for clarity and concision.)
Dan Garisto: What is the Standard Model and why do particle physicists want to break it?
Stefania Gori: The Standard Model of particle physics is a theory that describes the known forces of nature—electromagnetism, the weak interaction, and the strong interaction. The three forces of nature. [Editor’s note: Minus gravity.] And it's a very successful theory, because it is theoretically self-consistent, and it is very successful in describing data. So far, we have many measurements of elementary particles, and overall, they seem to agree with the Standard Model prediction.
Now, of course, in all our experiments, we try to see if there is physics beyond the Standard Model or if there is something that is unexplained. And the reason we are trying to do that is that we know there are many questions in particle physics that are not answered by the Standard Model. So we are trying to understand, indeed, how to answer these questions, and if the Standard Model is a full theory of nature or if there is something ‘beyond.’
DG: Before Wednesday [Editor’s note: 4/7/21], what would you have guessed is the most likely way that the Standard Model would break due to some new evidence in particle physics?
SG: We know from measurements that the universe is composed of some additional matter called dark matter that doesn't interact directly with electromagnetism. Dark matter cannot be a particle belonging to the Standard Model. Then there are other questions, like the hierarchy problem, that is, basically understanding why the Higgs particle is so light, if compared to the Planck scale.
Then we have the question about the baryon-antibaryon asymmetry—we'd like to understand why in our universe we have more matter than antimatter—we have the question about how to generate the neutrino masses, we need something beyond the Standard Model to understand neutrino masses. Theoretically, we have many open questions that we don't know the answers to. And I think, based on this question, ‘How to break the Standard Model?’, I am very interested in the dark matter story. So I am really hoping that, sooner or later, we'll discover a new particle that is describing and is giving us an answer to dark matter.
And these can be actually connected to other open problems in particle physics, or like the baryon-antibaryon asymmetry or the hierarchy problem. So all of these are guiding principles to understand how we can eventually break the standard model or how we can discover something beyond.
DG: It's interesting because so many of these things you've mentioned—dark matter, baryon-antibaryon asymmetry, etc.—I feel like they come from areas besides particle physics. They come from cosmology and astrophysics, but obviously, they inform what particle physicists are doing and how they conceive of physics beyond the Standard Model. They sort of set goals. Would you agree with that?
SG: Yeah, there is a really strong connection between the two fields of particle physics and astrophysics. There are other questions where there is a little bit less connection. But definitely, the fields—particle physics on one side, and astrophysics and cosmology, on the other side—are closely, closely connected. And this is also the beauty of the field, if you want, that one field informs the other one and vice versa.
DG: Given the results on Wednesday, does that change your view of where beyond the Standard Model physics might be located?
SG: Broadly speaking, the result on Wednesday is quite exciting, and very, very interesting. And I'm really looking forward to learn more about possible implications and also in the future new experimental or theory results. Having said that, it will be interesting to see how these experimental results can be put in a broader context of open questions in particle physics. Obviously, all the questions or the open questions in particle physics that I mentioned before, are still there. And, in the sense not incredibly much has changed for these questions, and I think it is exciting to see how this new measurement of g−2 can be put in a context.
DG: If you had to bet, what percent chance do you think it is that we're seeing a real signature of BSM physics at g−2? Just to put you on the spot.
SG: Yeah, that's hard to say. I would like really to be excited and to bet a lot on that. It's hard to say, obviously, so I'm not sure how to put a number on this. Sorry.
DG: Maybe I can rephrase the question. If data from the next experimental runs reaches five sigma, what kind of number would you put on that? Would you give it even odds?
SG: Five sigma, as you say, is the number of sigmas that we use to claim a discovery. So in that sense, taken at face value, five sigma would be a discovery of something beyond the Standard Model. That, of course, would give us more confidence that there is something beyond the Standard Model. Personally I would like to see, more experimental results for g−2 as well as additional theory developments in the calculation of g−2. Both directions should go hand in hand.
DG: How do you think about theoretical uncertainties, especially in the light of the BMW result?
SG: So g−2 has several contributions as predicted by the Standard Model, and one of these contributions is called the hadronic vacuum polarization. This contribution is being computed using two different techniques: One technique is the lattice type of calculations and the other one is a different technique that is based on data, or what we call the r-ratio. There is a Theory Initiative that formed several years ago with a goal of facilitating the communication between different groups involved in these calculations. Last June, this Theory Initiative with this working group came up with the first publication.
In that white paper, they show that actually, the r-ratio technique is predicting a value for the hadronic vacuum polarization that is more precise than the lattice prediction. And in the lattice prediction, the central value is closer to the experimental measurements, but with larger uncertainties. Then what you do is to combine these two techniques, and make the prediction for g−2. Of course, this prediction is dominated by the r-ratio technique that is more precise.
This is in a result that has not been included in that white paper—there’s been a lattice calculation, the BMW calculation, that basically is claiming smaller errors, a smaller uncertainty with a central value that is closer to the measurement. So there is quite a bit of discussion ongoing, between the people involved in this BMW collaboration and basically everyone in the working group of this g−2 theory initiative to understand this new lattice result.
DG: Assuming two equally uncertain lattice and r-ratio results, do you have a bias toward either method? The ab initio calculations from lattice—would you trust that more than you would trust the e+e- data? Or is it the other way around?
SG: Not really. It's great to have two completely different techniques to compute that quantity. We should obviously take both of them super seriously and understand better, both of them to see what comes out. But I mean, it's great to have two different directions to compute this quantity, because actually, this contribution—the hadronic vacuum polarization—is the contribution that has the most part of the uncertainty.
DG: Alright, let's talk about phenomenology. I know you work on a lot of Higgs models, and one of the things that struck me—I think I was talking to Gino Isidori about this. He seems to think that it is very likely any kind of lepton universality breaking is connected to the Higgs because of the mass differences between generations. Do you think that's correct? Do you have thoughts about that? If there is new physics to be found at g−2 is there likely to be a connection to the Higgs?
SG: There could be. So actually, there are models that are making this connection that you say between g−2 and the Higgs or more generically extended Higgs sectors—theories beyond the Standard Model where you have not only the Higgs of the Standard Model, but additional scalars, additional Higgs bosons. So this is an open possibility that is quite exciting and there are quite a few works in the literature. If you just think about the Standard Model, the Higgs is the only particle that is distinguishing between the several fermion generations. The Higgs is the only particle that is telling you the difference between a muon and an electron.
DG: So far.
SG: That's right. So, I think this is a very fair and interesting question. Of course, this is not the only possibility in the sense that there are theories beyond the Standard Model that can address this g−2 anomaly in different ways that are not necessarily connected to the Higgs particle or having new scalars. For example, there are models where you have new forces, new gauge interactions that are, giving us the extra contribution to g−2. From my theory perspective, this is very interesting to study, in general, what one would need to address g−2, or eventually also, other anomalies that we have.
DG: What kind of biases do you have towards theories that address g−2? What do you like? What do you think sort of would be the most likely explanation?
SG: There are models that I worked on that are kind of simple—simple in the sense that you take the Standard Model, and maybe you add only one additional particle in such a way to address this anomaly. And just based on simplicity, one can say this could be a “natural” explanation of g−2. So, for example, there is a simple theory to have the Standard Model plus a new Z'. So, a new gauge boson that couples to muons and eventually also the taus, and that could address the g−2 anomaly, if the Z’ mass is relatively light, so below the GeV scale or so.
There are simple theories that can address g−2 while still being consistent with all other data that we have. And this is quite interesting, because if you take g−2 the measurement at face value, what you learn is that you need to have a new physics particle that is relatively light, it cannot be incredibly, incredibly heavy. And then you might wonder if this is not already scouted by other data and other searches. It's very interesting to know that there are simple theories that can address g−2 with a relatively light new particle that are not really probed by any other experiment. And this is a quite exciting,
DG: But you could also have a heavy particle that is just not going to have a strong coupling to electrons because it's suppressed due to the mass, right?
SG: Correct. Yeah. The point is that if you want to have a sizable contribution to g−2, this particle cannot be super, super heavy. It cannot be much beyond the electroweak scale, otherwise your new physics contribution would be too small.
DG: Oh, I see. When you said heavy I was assuming you were saying nothing above, like, 100 GeV.
SG: No, no. I mean hundreds and hundreds of TeV, so you need something at around the electroweak scale, roughly speaking.
DG: It struck me that people had their picks—some people like leptoquarks, some people like Z’s. And I've seen some of the papers on arXiv, published right after the announcement, proposing winos and binos. And I guess I wonder, are there models that make people say, “Well, this could be possible, but really this is terrible to work with. I don't know that we want this to happen, even though it'll be new physics.”?
SG: You might have, as a guiding principle, the principle of simplicity. And then some of the explanations could be less appealing than others, because they are less simple in the sense that you start to add a lot of particles that are not particularly motivated. This depends on what is your guiding principle and how much you believe in simplicity. Now, there are several frameworks that maybe are not as simple in the sense that you are adding much more than one particle, but nevertheless, they are quite appealing. For example, in the framework of supersymmetry, where obviously, you add many more particles to the Standard Model, because you have all the supersymmetric particles, but is nevertheless very, very well motivated.
So it depends really on what are your guiding principles to make this judgment. Personally, all these theories that we mentioned—these leptoquarks, or this Z’ or supersymmetric explanations could be all possible. I don't feel like having too big of a theory bias. Of course, if you start to put together several of the anomalies in data, for example, g−2 and also flavor anomalies, it can be more complicated to address all of them, using just one theory. But this, again, is a little bit of theory bias. And I think what is important for us as theorists is to understand what are the theories that allow us to eventually address this anomaly and then make predictions for experiments to test these theories. And then to see what really nature is telling us.
DG: So, okay, fair point, I agree that maybe it's good for theorists to not be biased. But we just had the European strategy update and Snowmass will resume next year. Money is not unlimited, and we have to decide what to look for. I believe the highest priority item for the European strategy update is a Higgs factory of some kind, whether it's the ILC or some other new collider. Do the Muon g−2 results change what the highest priority is for Snowmass? Should they?
SG: So, obviously, g−2 is very important. And as I mentioned already several times, this is an exciting new result. And this is strengthening the open question that we had since the time of the Brookhaven measurement. I think in this context, during the Snowmass process, we should really understand what are the prospects for understanding the physics that could be involved in g−2, or eventually new experiments that can test this quantity, because this is an open question. At the same time, thinking about Higgs physics, the Higgs particle is certainly the last particle that we have discovered and is the least known particle among the particles of the Standard Model. Of course, there are neutrinos that are also not well understood, but having said that, I think understanding better the Higgs particle should be one of the highest priorities.
DG: Just for you personally, do you have three, four top priorities for experiment?
SG: Yes. So here you can see my bias if you want. Personally, I've been working a lot on Higgs physics and in general high energy physics, as well as lower energy, dark matter physics. And I think it would be very important to have a program that is really an experimental program that is going in these two directions. One is the high energy, so having high energy colliders that, first thing they test better and better the Higgs particle, and then they have access to higher energies, so to eventually discover heavier new degrees of freedom. This is one direction and the other direction is the low energy direction: Making experiments for precision experiments that can eventually tell us something about new physics in an indirect way, or eventually discovering light, new degrees of freedom. And oftentimes, these are much smaller experiments that are nevertheless contributing a lot to our understanding of what happens at lower energies.
DG: Obviously, a lot of these new experiments are going to take some time to happen, if they happen at all, and I know some people have been proposing a muon collider, which is a little far out there but interesting nonetheless. But in the meantime, the LHC is starting back up next year. Is there anything from this next run you're particularly looking forward to that they will have capability to do or just have more data that they didn't have before?
SG: There are many things actually. Firstly, there are many questions for the Higgs particle that would be nice to understand better. As we talked about last summer, we had the first evidence for the Higgs coupling to muons. And so for the first time, we had evidence for the Higgs coupling to the second generation. I think this is pretty interesting and this is something that, soon, we'll have a much better understanding in the sense that soon, if indeed the Higgs couples to muons, as you would expect, we have a discovery of this new decay.
Just to bring an example to say that there are several characteristics of the Higgs boson that we will understand much better in the coming advance of the LHC. We'll have new discoveries. So we expect to have a discovery [Editor’s note: ‘Discovery’ in this context means that the data are extremely statistically significant—unlikely to be a fluke.] for the Higgs going to two muons. We can have better measurements or searches for this possibly CP violating component to the Higgs because so far we don't know if the Higgs is a combination of a CP-odd and a spin even particle. So there are many questions associated to the Higgs that we will learn much better in the coming years of the LHC.
DG: I want to ask you about other anomalies: g−2 is maybe the biggest one, but there's also the KOTO anomaly, and there's the series of anomalies at LHCb. How do you think about those anomalies when you decide to write a phenomenology paper that explains them? Are you cherry-picking? How do you pick anomalies to explain?
SG: From time to time I write papers on anomalies. This is not something I do super frequently, but sometimes it's just exciting to understand what anomalies in data can tell us. In general, it can be hard to explain many of those at the same time. Obviously, it's much easier if you take a set of anomalies—for example, the LHCb anomalies—and try to explain them.
The papers that I've been writing on anomalies, were focused on some specific set of anomalies. The anomalies that you mentioned on the LHCB, on lepton flavor universality are super interesting. And this is something indeed, that I wanted to mention that I would be very curious to learn more in the coming years with more data from the LHCb collaboration.
DG: Yeah, I just wanted to briefly ask about it, because you mentioned light dark matter. You've written about axions. Why axions?
SG: Initially, they were proposed to address the strong CP problem. We have the QCD axion, and that is strongly theoretically motivated. And what is pretty interesting is that axions can be also a dark matter candidate. Axions are pseudoscalars with a mass that is protected by an approximate symmetry, and there are plenty of theories that do predict these type of new particles, even beyond the initial motivation of the strong CP problem. So, these are some particles that theorists love. We are trying to understand more and more theoretically, and also trying to think about new measurements and new experiments that can test these particles.
DG: What are you most excited for in this upcoming year?
SG: In terms of experimental data, I think, the most exciting data are from g−2 and these anomalies from the LHCb collaboration, and then there are a few anomalies also from LHC, that are kind of interesting. Beyond anomalies, there are these very important open questions in particle physics that really excite me. One is the question about dark matter. And the other one that I am actually presently working on is the baryon-antibaryon asymmetry problem. These are theoretical questions that are there and are independent of the anomalies. Being a theorist these days, it's nice to follow anomalies and to try to understand what they are telling us. And at the same time, there are deep questions that are always there.